Introduction
Point of Care Ultrasound (PoCUS) is the use of portable ultrasonography to answer specific, focused clinical questions at the bedside. It is an extension of both our clinical acumen and physical exam. PoCUS is commonly used in adult trauma cases as part of the focused assessment with sonography in trauma (FAST) examination. In undifferentiated and hemodynamically unstable patients, PoCUS can rapidly identify intra-abdominal free fluid which can expedite management and disposition decisions. When ascites is suspected, PoCUS may be used to confirm the diagnosis and guide further management. In recent years, the use of PoCUS has expanded to include pediatric patients as well.
Why Ultrasound?
PoCUS is superior to physical exam in detecting intra-abdominal free fluid. In addition, PoCUS can be performed quickly at the bedside without exposure to ionizing radiation. Physical exam alone is a poor test for the detection of peritoneal free fluid, with an overall accuracy of 58% [1]. Studies have shown that as little as 10-50cc of peritoneal free fluid can now be detected with ultrasound. In the hands of emergency doctors, volumes of 100-200 mL of fluid can be routinely detected at the bedside [2,3]. These numbers hold true for trauma patients as well. In 2007, Soyuncu et al. showed emergency physician performed ultrasound detected intraperitoneal hemorrhage with a sensitivity of 86% and specificity of 99% while physical examination reported a sensitivity of 39% and specificity of 90%, respectively [4].
The use of ultrasound in the child who has sustained trauma requires special mention. In the setting of the unstable trauma patient it is critical to identify the cause of decompensation as early as possible as it helps guide and prioritize management decisions, including further diagnostic workup and early disposition from the ER. The focused abdominal sonography in trauma (FAST) exam is both sensitive and specific in critically ill, hypotensive pediatric trauma patients [5]. It is best used to screen for significant intra-abdominal injuries requiring emergent transfer to the operating room in the unstable patient. In the stable patient, the utility of the FAST is debated and the sensitivities range from 25-80% for the detection of all intra abdominal injuries, though it performs better in those injuries with hemoperitoneum [6]. Performing serial FAST as well as the combining findings with clinical exam and laboratory investigations can increase the sensitivity of the FAST examination [7, 8, 9]. While CT remains the gold standard screening tool for intra-abdominal injuries, the use of the FAST exam may have the ability to decrease CT use in pediatric patients.
The FAST scan can be a challenging scan to master as adequate sonographic windows may be difficult to obtain and multiple areas must be investigated under challenging conditions. Studies show the false negative rate of scans decreases with increasing physician experience. Deliberate practice is key to attaining proficiency [10].
Summary
Ultrasound to identify joint effusions is widely used to assess those children presenting with a limp, refusal to weight bear or with a painful or swollen joint. PoCUS can be used to rapidly evaluate these children at the bedside thereby expediting management. This may include early referral to orthopedics. The use of PoCUS has also been shown to improve the ability of physicians performing arthrocentesis on joint effusions. PoCUS improves accuracy and decreases patient pain, without increasing the length of time required to perform the procedure [11,12].
Pitfalls
In all joints, it is important to assess both the affected joint, and the contralateral side, as a small amount of fluid within the spaces can be normal. In addition, cartilage can appear hypoechoic within these spaces, but will be non-compressible [1]. Finally, fluid within an effusion can be simple (anechoic) or complex (heterogenous in echogenicity) depending on what caused the effusion. Examples of potential complex effusions include hemarthrosis with clots or loculated septic arthritis [1, 3]. Synovitis can mimic a complex effusion, but can be differentiated with the addition of color to look for low grade venous flow.
Ankle Anatomy Review
The area where an effusion in the ankle collects is at the intersection of the tibia and the talus (Figure 15). There is a fat pad (the anterior fat pad) at the junction of these two bones. Superficial to the boney surfaces, the tibialis anterior tendon is found.
Figure 15: Anatomy of the ankle joint.
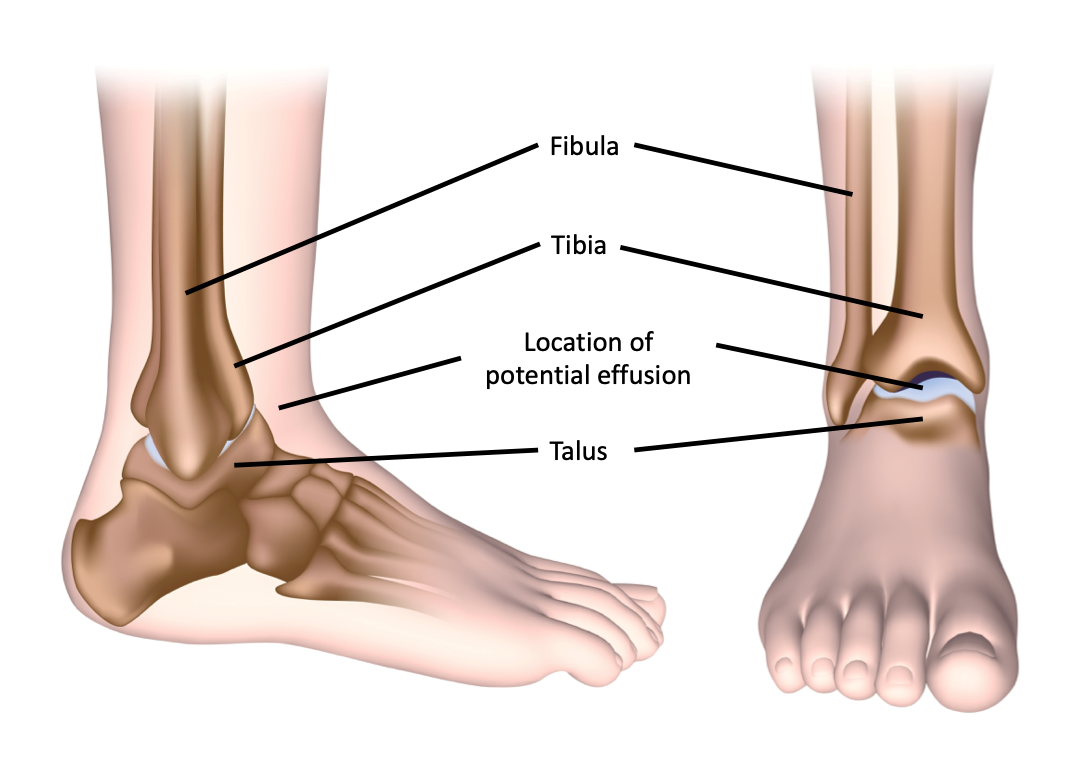
Technique
- Position the patient supine.
- Have the patient flex their knee and place their foot on the bed to place their ankle in dorsiflexion [1].
- Place the linear array probe along the longitudinal axis of the tibia at the joint line between the tibia and talus [1] (Figure 16).
Figure 16: Probe position for ankle ultrasound.
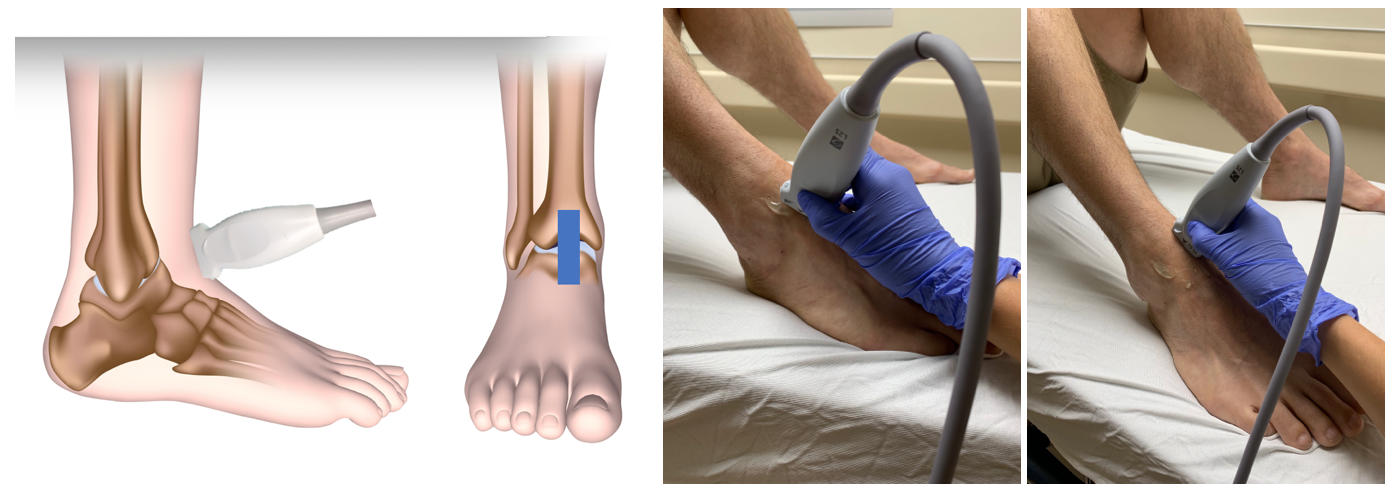
- Identify synovial space at the junction of the talus and the tibia.
- Assess for presence of joint effusion.
- Repeat on the contralateral joint.
What am I looking at?
Figure 17: Labelled normal ankle ultrasound. Note that physiologic fluid and cartilage can appear similarly, so differentiate them with compression of the structures. Fluid will compress, cartilage will not.
Tibia
- Hyperechoic line cephalad.
Talus
Tibialis anterior tendon
- Fibrillar hyperechoic structure in near field.
Anterior fat pad
- Immediately superficial to the synovial space.
Anterior talotibial recess or synovial space
- Superficial to the bony surfaces of the joint.
What is normal?
On ultrasound of the ankle, you will see the tibia extending cephalad and the joint line between the tibia and the talus (Video 4). Within the joint space, near field to the bony structures, there may be a small amount of physiologic fluid, and an anterior fat pad. As in the other joints, physiologic fluid will follow the contours of the bone, and have a more concave appearance (Figure 18).
Video 4: Normal ankle ultrasound.
Figure 18: Normal ankle ultrasound.
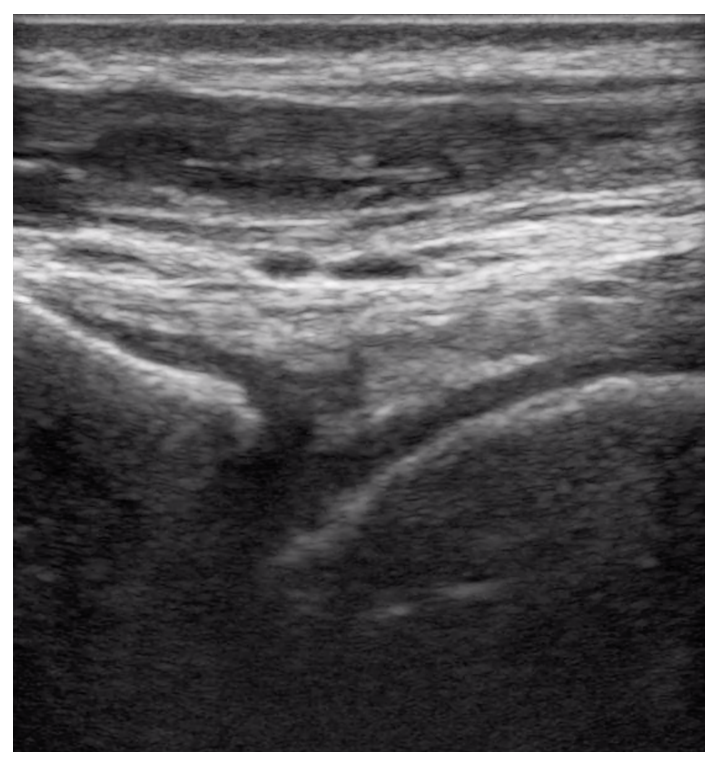
What is NOT normal?
An ankle effusion is characterized by a convex shaped hypoechoic fluid collection anterior to the tibial and talar joint (Figure 19). In the ankle, unlike in the hip, there is no specific measurement for a positive effusion – the important feature to look for is the convexity of the collection (Video 5).
Figure 19: Positive ankle effusion.
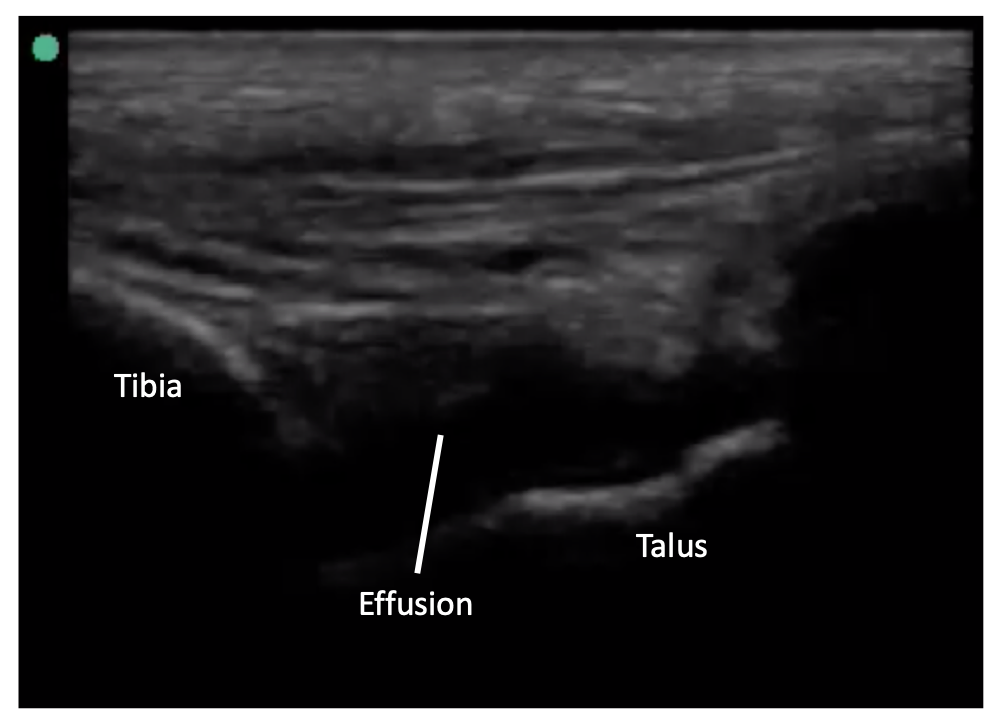
Video 5: Ankle joint effusion. Again, note the convex appearance of the fluid.
Knee Anatomy Review
The knee is made up of the articulating surfaces of the femur and the tibia, with the patella lying anteriorly. The quadriceps tendon inserts on the patella, and the patellar tendon extends distally to the tibial tuberosity. The fluid within the synovial capsule is continuous with the suprapatellar bursa. The fat pads found within the knee joint include the quadriceps fat pad and the pre-femoral fat pad (Figure 10).
Figure 10: Anatomy of the knee joint.
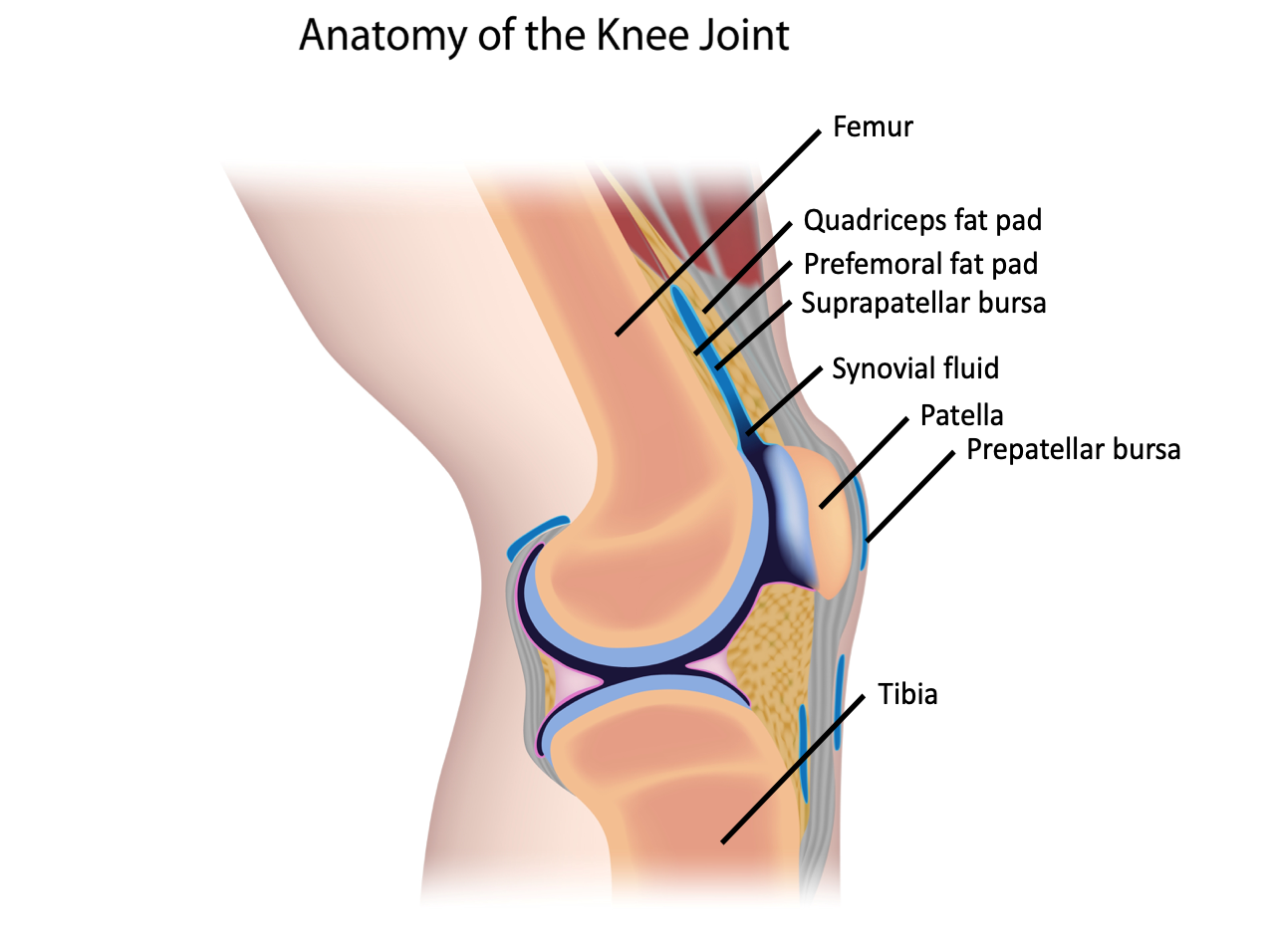
Technique
- Position the patient supine.
- Use a towel roll to place the affected knee in 20-30 degrees of flexion [1] (Figure 11).
Figure 11: Probe position for knee ultrasound
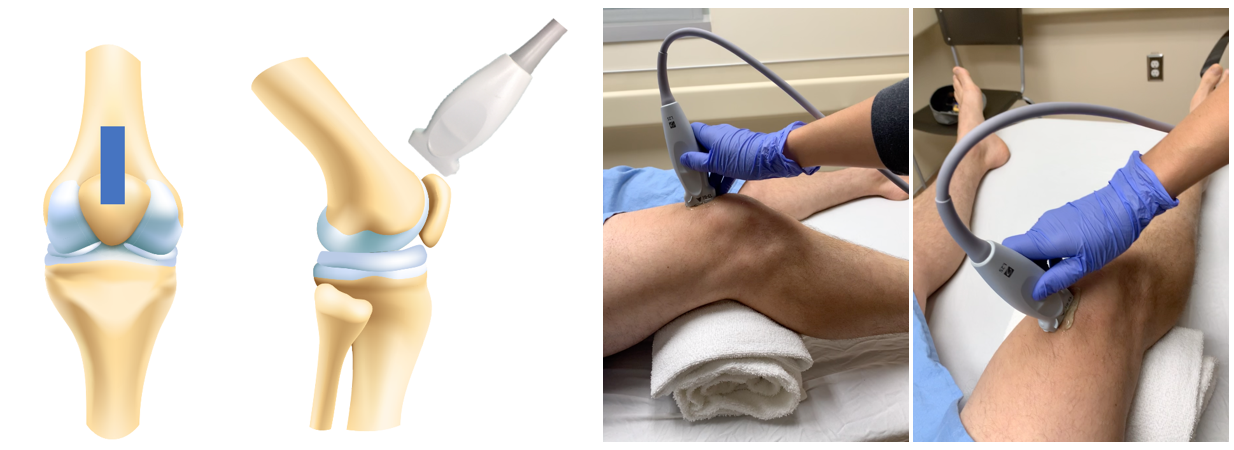
- Place the linear array probe longitudinally in the sagittal plane on the patella [10].
- Identify the patella and scan proximally to assess for effusion within the suprapatellar recess [10].
- Assess for presence of joint effusion.
- Repeat on the contralateral joint.
What am I looking at?
Figure 12: Labelled normal knee ultrasound.
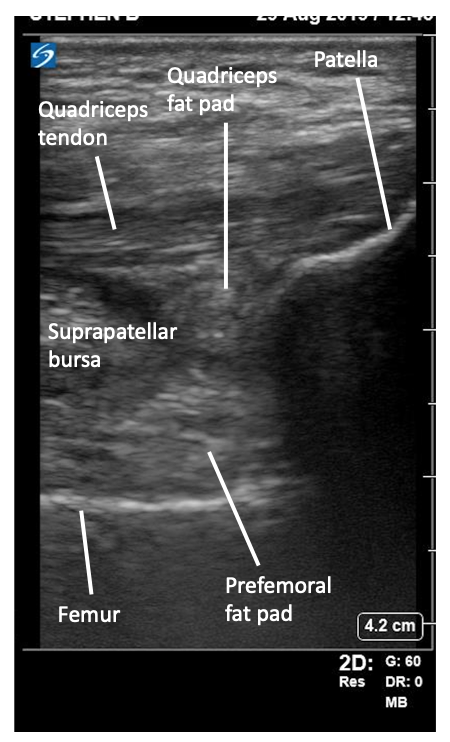
Patella
- Hyperechoic line in caudal field of view.
Femur
- Hyperechoic line in cephalad field of view.
Quadriceps tendon
- Hyperechoic fibrillar structure in near field.
Fat Pads
- Pre-femoral fat pad – hyperechoic soft tissue collection just anterior to femur.
Quadriceps fat pad – hyperechoic soft tissue collection just inferior to quadriceps tendon.
Suprapatellar bursa
- Hypoechoic potential space.
Prepatellar bursa
- Hypoechoic area superficial to patella.
What is normal?
Visualization of the knee joint in this view will show you the patella caudally and the femur extended cranially (Video 2). The quadriceps tendon will be a fibrous structure running in the near field (Figure 13). The suprapatellar bursa should be less than 2mm thick [1].
Video 2: Normal knee ultrasound.
Figure 13: Normal right and left knee ultrasound.
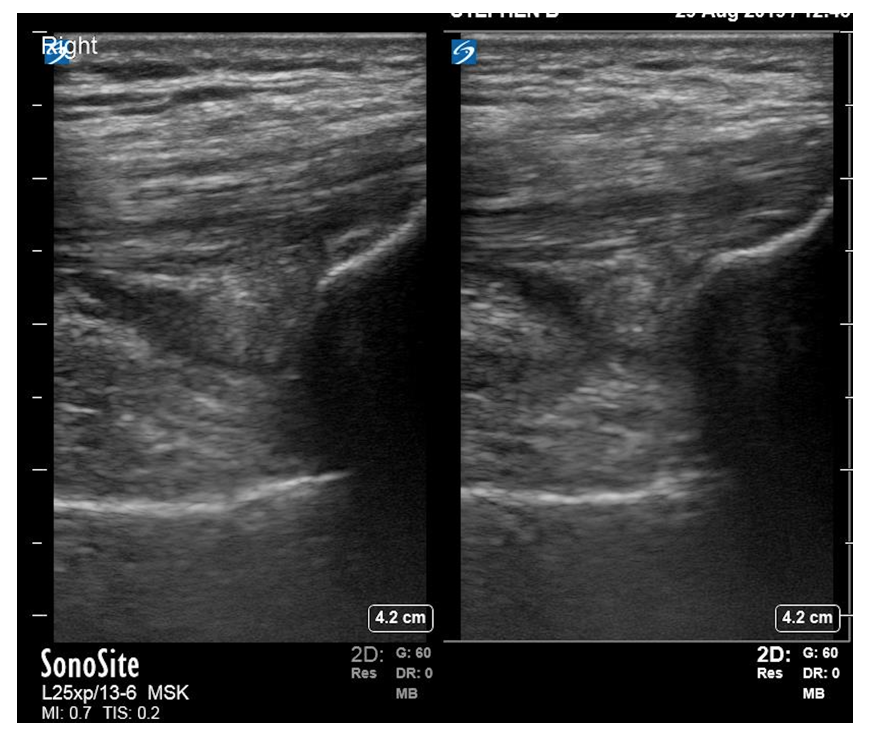
What is NOT normal?
Fluid will collect in the suprapatellar bursa, found in between the prefemoral and quadriceps fat pads (Video 3). The ultrasound is positive for a knee effusion by a collection of hypoechoic fluid which is >2mm thick [1] (Figure 14). If you are not seeing any fluid collection, flexion of the knee can increase the fluid in the suprapatellar recess [13]. In addition, fluid can collect on both the medial and lateral side of the suprapatellar region, therefore scanning these areas can help identify fluid collections. To do this, slide the probe laterally and medially within the sagittal plane to identify hypoechoic fluid collections. This can help with performing ultrasound guided arthroscopy of the knee, although that is outside the scope of this module.
Video 3: Knee joint effusion. Note the fluid collection between the quadriceps fat pad and quadriceps tendon superiorly and the prefemoral fat pad inferiorly.
Figure 14: Positive knee effusion.
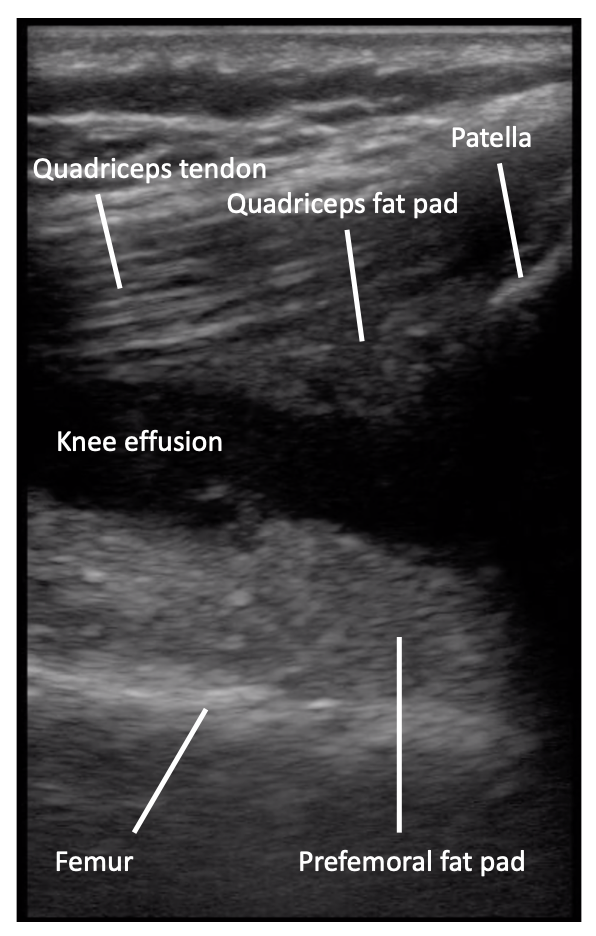
Hip Anatomy Review
The hip joint is a ball and socket joint, comprised of the femoral head and neck and the acetabulum (made up of the ischium, pubis and ilium). The joint capsule surrounds these structures and contains synovial fluid (Figure 4).
Figure 4: Anatomy of the hip joint
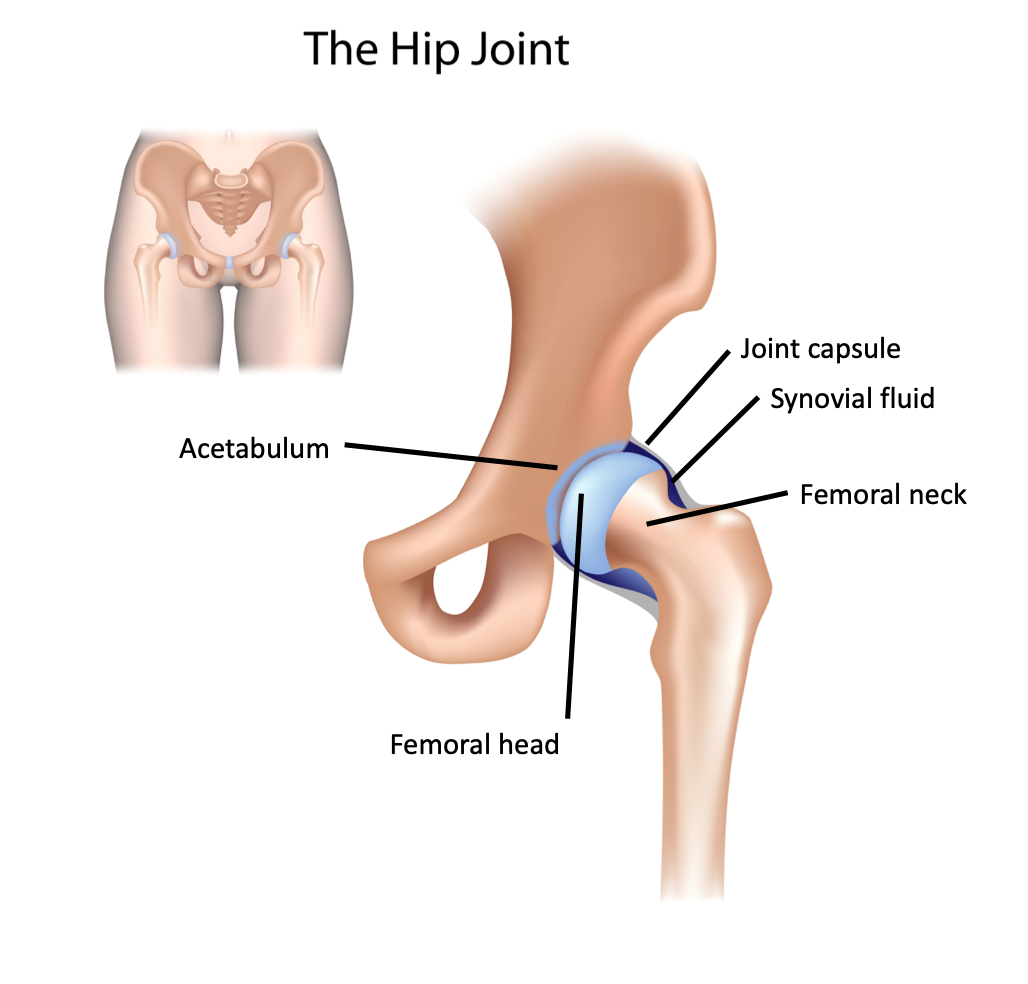
Superficial to the bony structures, we find the musculature of the pelvis and the hip. Those that are relevant to us for the purpose of scanning for hip effusions include the iliopsoas, quadriceps and sartorius. These muscles are found superficial to the joint (Figure 5).
Figure 5: Hip musculature
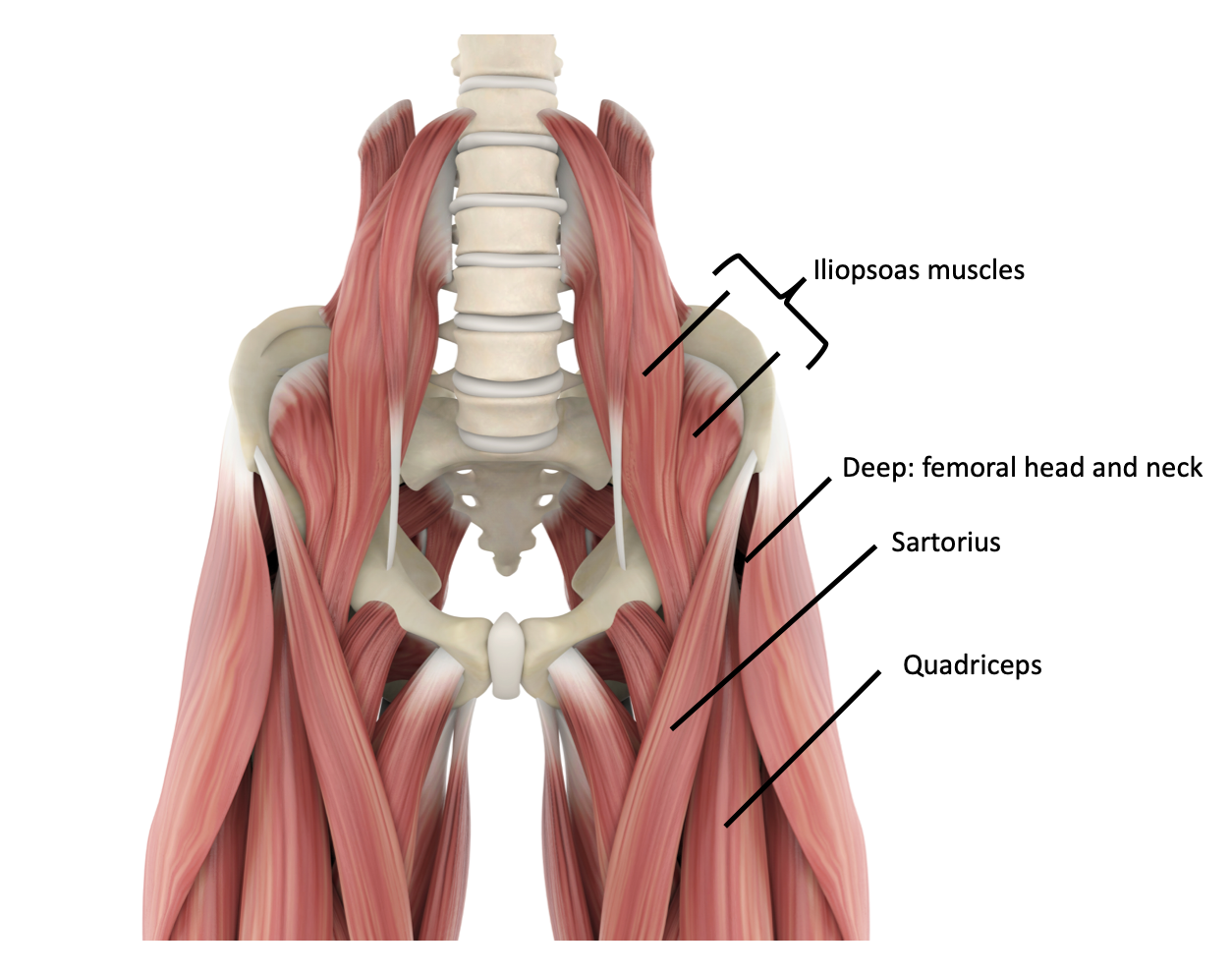
Technique
- Position the patient supine, with the affected hip in a neutral position [4].
- Place the linear array probe below the femoral crease, with the probe at a 45-degree angle and pointing posteriorly on the patient: parallel to the neck of the femur (Figure 6).
Figure 6: Probe position for hip ultrasound
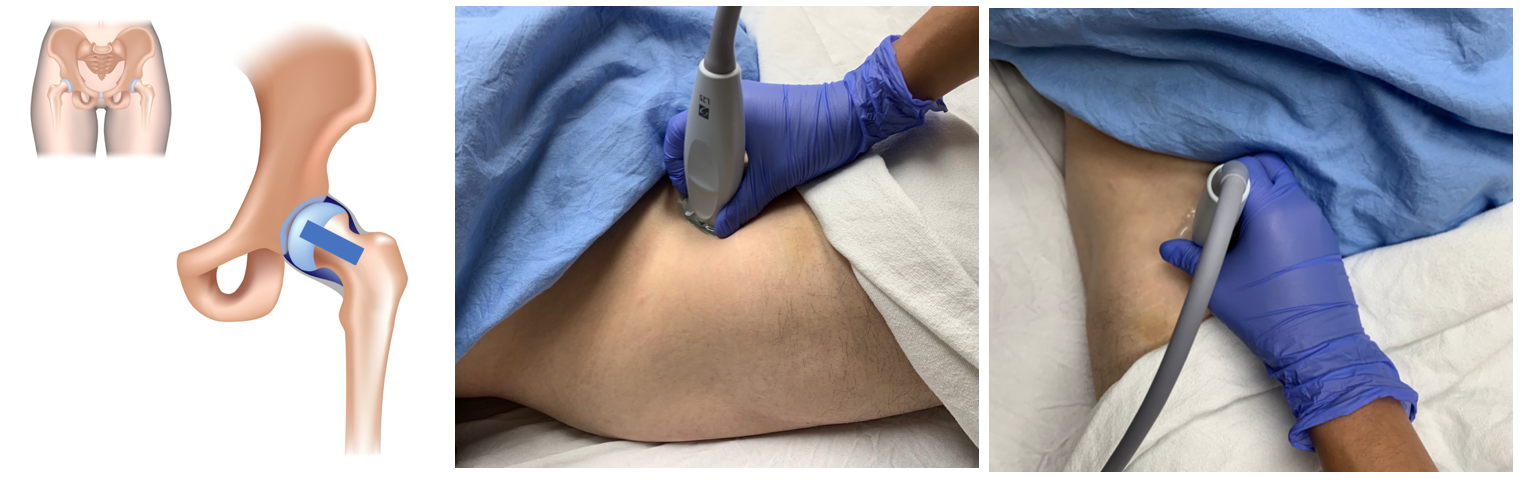
- Identify the femoral neck, femoral head, iliopsoas and synovial space in between [7].
- Assess for presence of joint effusion.
- Repeat on the contralateral joint.
What am I looking at?
Figure 7: Labelled normal hip ultrasound.
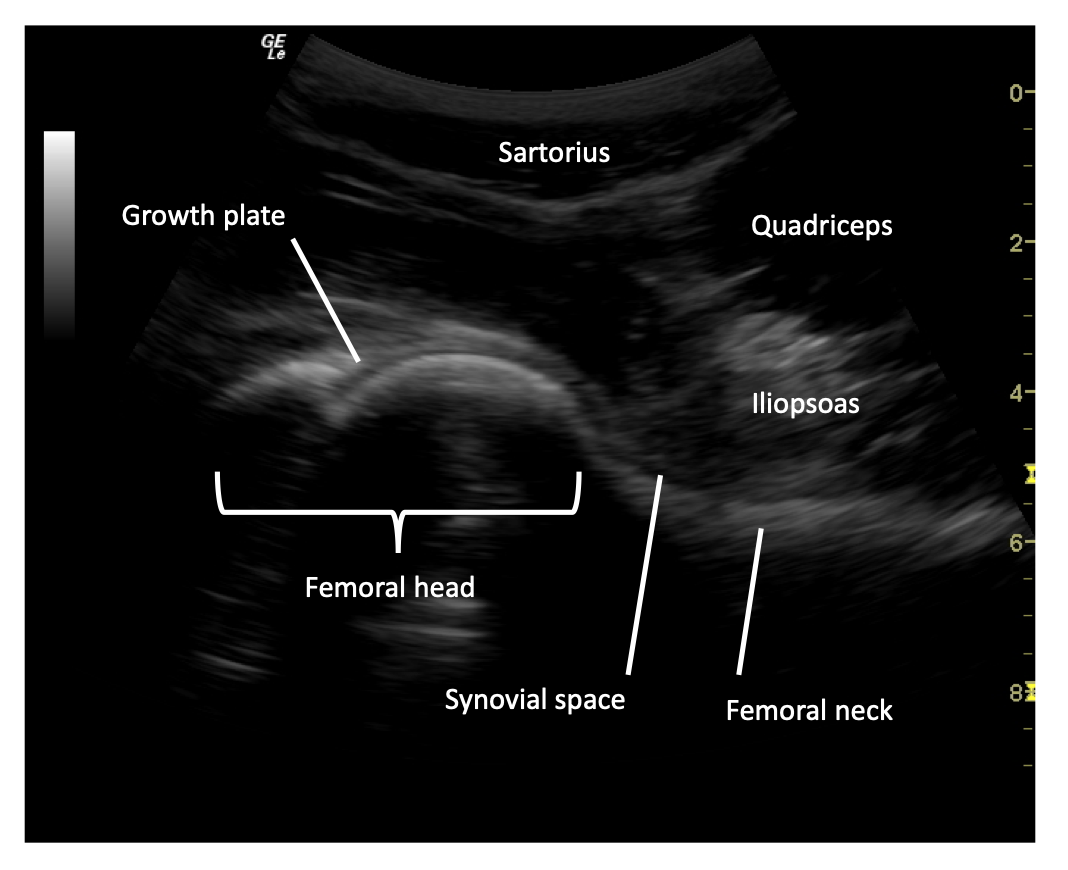
Femoral neck
- Hyperechoic horizontal line.
Femoral head
- Curved hyperechoic line.
- Physis or growth plate can be seen as a notch or hypoechoic break in the femoral head [7].
Muscles
- Iliopsoas muscle is superficial to the synovial space.
- Quadriceps and sartorius are the most superficial structures in the field of view.
Synovial space
- Space between the femoral neck and the iliopsoas muscle.
What is normal?
Normally, synovial fluid will follow the contours of the joint itself, in this case, the femoral head and neck. The size of a fluid collection in the hip is measured from the anterior surface of the femoral neck to the posterior surface of the iliopsoas muscle [4]. The space should be <5mm and within 2mm in comparison to the unaffected hip (Figure 8).
Figure 8: Normal hip ultrasound.
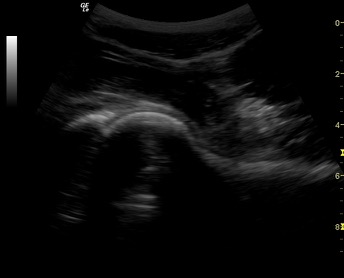
What is NOT normal?
The presence of a joint effusion in a hip is defined as a space between the anterior surface of the femoral neck to the posterior surface of the iliopsoas muscle of >5mm or >2mm difference in comparison to the unaffected side (Figure 9). An effusion will classically have a convex appearance (Video 1) in comparison to the normal concave appearance of the synovial fluid in a joint without an effusion.
Figure 9: Positive hip effusion.
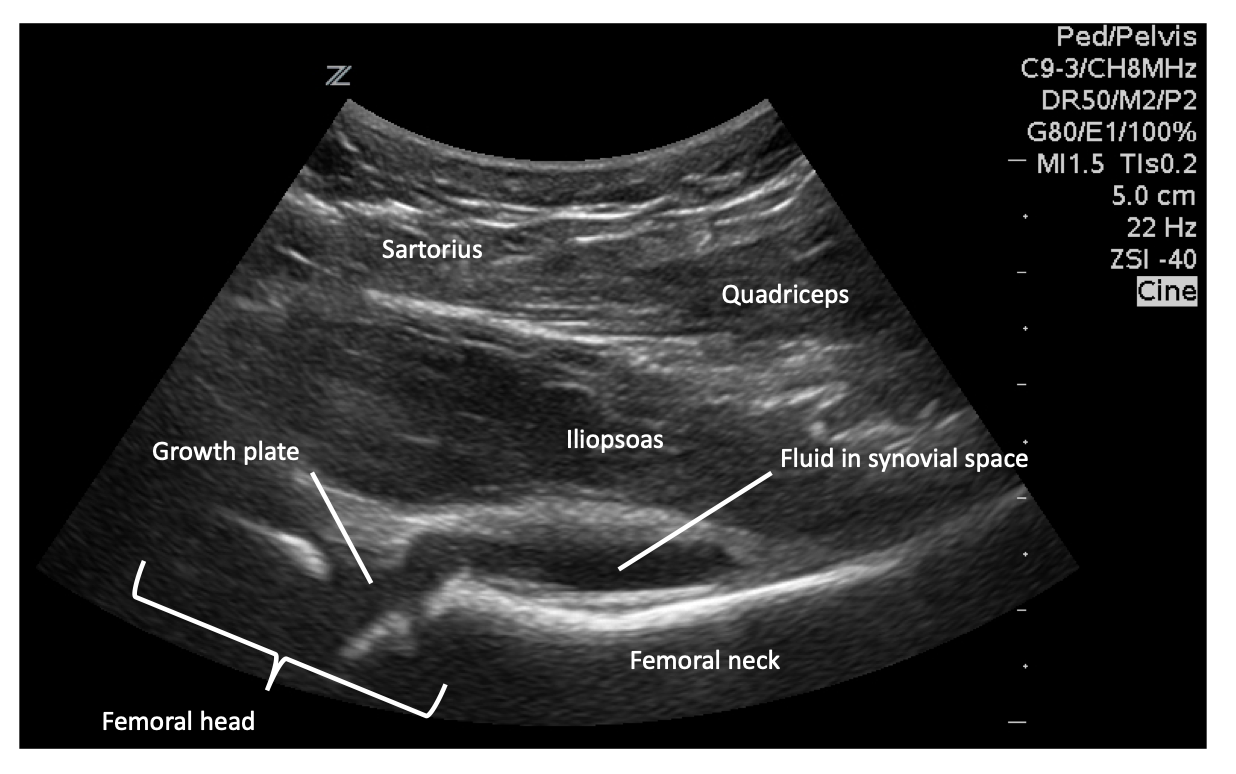
Video 1: Hip joint effusion. Note the convex appearance of the fluid on the image on the right.
Sonoanatomy Review
Normal skin and soft tissue have many layers (Figure 1). The most superficial structures are the epidermis and dermis, which appear as if one hyperechoic structure. Deep to this, you will find subcutaneous fat, which is hypoechoic and globular. Blood vessels, nerves and lymph nodes are found within the hypodermis, and are varying in their echogenicity. Deep to these structures, the muscle is found beneath a hyperechoic layer of fascia. Muscle is seen as highly organized hypoechoic and striated fibers.
Figure 1: Anatomy of normal skin
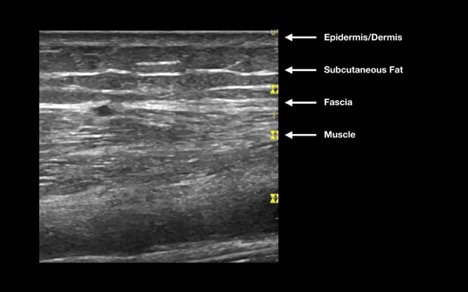
Tendons are visualized as hypoechoic fibrillar organized structures, whereas fat pads are also hypoechoic, but are more homogenous in their appearance (Figure 2). Finally, smooth hyperechoic bone cortex may be seen as the deepest layer.
Figure 2: Anatomy of a normal joint
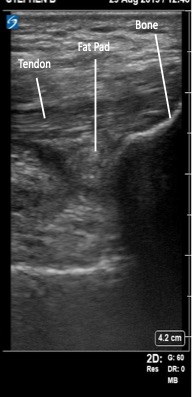
Tip:
Scan with dual screen functionality
In order to facilitate comparing an affected joint with the contralateral side, it can be useful to use the dual screen function on the ultrasound machine.
- Choose “More Controls” from the main screen, found at the bottom right corner (Figure 3a).
Figure 3a: Choose “more controls”
- Select “Dual” from the options screen (Figure 3b)
Figure 3b: Choose “dual”
- Touch the screen on either side to start scanning. When ready to switch, freeze the area of interest on the screen and then touch the opposite screen to begin scanning the opposite side (Figure 3c).
Figure 3c: Toggle between screens by touching the desired side
- Below is an example of a split screen view (Figure 3d).
Figure 3d: Dual screen example